Thank you for visiting nature.com. You are using a browser version with limited support for CSS. To obtain the best experience, we recommend you use a more up to date browser (or turn off compatibility mode in Internet Explorer). In the meantime, to ensure continued support, we are displaying the site without styles and JavaScript.
Scientific Reports volume 12, Article number: 15294 (2022 ) Cite this article Rare Earth Boride Powder

In the present work, we report on the microstructural and optoelectronic properties of SnOx thin films deposited by a reactive radio frequency magnetron sputtering. After SnOx growth by sputtering under O2/Ar flow, we have used three different treatment methods, namely (1) as deposited films under O2/Ar, (2) vacuum annealed films ex-situ, and (3) air annealed films ex-situ. Effects of the O2/Ar ratios and the growth temperature were investigated for each treatment method. We have thoroughly investigated the structural, optical, electrical and morphology of the different films by several advanced techniques. The best compromise between electrical conductivity and optical transmission for the use of these SnOx films as an n-type TCO was the conditions O2/Ar = 1.5% during the growth process, at 250 °C, followed by a vacuum post thermal annealing performed at 5 × 10–4 Torr. Our results pointed out clear correlations between the growth conditions, the microstructural and optoelectronic properties, where highly electrically conductive films were found to be associated to larger grains size microstructure. Effects of O2/Ar flow and the thermal annealing process were also analysed and discussed thoroughly.
Transparent conductive oxides (TCOs) are imperative materials in various technologies requiring optical transparency and electrical conductivity. In addition to these two characteristics, TCOs combine a third property, namely a high chemical stability. More specifically, owing to its optoelectronic properties and its production scalability, tin-doped indium oxide (ITO) is a preferred TCO for industrial applications and it has been extensively employed as transparent electrodes for various solar cells technologies, light emitting diodes and flat panel displays1,2,3,4,5,6,7,8,9,10,11. However, researchers are working on the development of alternatives materials to ITO due to the Indium (In) supply challenges in the future. Tin (Sn), instead, is a low-cost material of much higher earth abundance than Indium (In). Two stoichiometric tin oxide compounds, namely SnO and SnO2, are known to be wide band gap oxide semiconductors with tetragonal litharge and rutile type structures, respectively. Tin in SnOx has two chemical states of Sn2+ for SnO phase and Sn4+ for the SnO2 one. The first is intrinsically p-type semiconductor while the second is n-type12,13.
In particular, SnOx has been largely investigated in applications of gas sensors, solar cells, transparent electrodes, and thin film transistors11,14,15,16,17,18,19,20. Moreover, in the past decades, SnO was the key material for anode materials21, coatings22, catalysis23, and precursors for the production of SnO224,25, because of its properties of gas-sensitivity and metastability to transform into SnO2 at O2-rich ambient. Recently, SnO has been drawn back into attention mainly because of the difficulty in obtaining high-quality p-type such as doped ZnO26,27, NiO28,29, Cu2O30. Previous studies show that the maximum hole mobility of SnO films is about 2.6 cm2/V s, fairly high among p-type conductive oxides, and it can be further improved via proper doping19. Those properties render SnO a promising candidate to be a next p-type oxide semiconductor for advanced optoelectronic devices. Several techniques have been used to grow SnOx films on various substrates, including reactive RF magnetron sputtering31, e-beam evaporation18, laser ablation25, and atomic layer deposition32. However, the prepared SnOx films are often mixed with some impurity phases, including metallic Sn, and intermediate oxides containing both 2+ and 4+ valences31,33,34. The reason is that SnO can decompose according to the disproportion at ion reaction even in the absence of oxygen at suitable temperature16,17. Consequently, deposition conditions and growth temperature are of great importance in the fabrication of single phase SnOx films.
In the present work, polycrystalline SnOx thin films were fabricated on quartz substrates by a two-step method, i.e., RF MS from high-purity SnO source target first and subsequent air and vacuum annealing treatments. The morphology, crystal phase, chemical composition, optical, and electrical properties of the obtained SnOx thin films were characterized by Grazing Incidence X-ray Diffraction (GIXRD), Scanning Electron Microscopy (SEM), Transmitting Electron Microscopy (TEM), UV–visible, and Hall effect measurement, as detailed in the experimental section. The depth profiling of the various elements was also investigated by time of flight-second ion mass spectroscopy (TOF-SIMS) throughout the thickness of the films. The results show in detail the effect of the oxygen flow and the temperature during the growth on the microstructure and eventually on the electrical conductivity and the optical transmittance. The optimized conditions were clearly defined and discussed thoroughly. The majority of the samples exhibit an average optical transmittance with more than 80% between 400 and 700 nm, while the highest conductive thin films are dense, with large grain size and without pinholes and/or cracks.
The SnOx thin films were prepared in two subsequent steps. The first step consists of the RF magnetron sputtering (manufactured by Torr) of a high purity SnO (99.99%) 2″- diameter target (Manufactured by Codex International) on 1″ × 3″ cleaned quartz substrates at two different temperatures, namely 100 and 250 °C, under different O2 to Ar flow rates ratios, namely O2/Ar = 0, 0.5, 1.5, 2.5, 4.5 and 7.5%. Each sample was cut into three 1″ × 1″ smaller samples. The second step consists of two different post deposition annealing at 400 °C for 1 h, one in air and the other one in a controlled moderate vacuum (with a constant Ar flow of 5 sccm) at 5 × 10–4 Torr.
The depositions conditions using RF magnetron sputtering were: 50 W power, 200 sccm of Ar flow and 30 min deposition time for all samples. The first batch was deposited at 100 °C, and the second batch was deposited at 250 °C. Prior the deposition, the base pressure of 5 × 10–5 Torr was first achieved, and the deposition pressure, which depends on the variable oxygen flow rate, varied from 4.4 × 10–3 to 5.1 × 10–3 Torr.
Several characterization techniques were employed to investigate the micro-structure, crystalline structure, optical transmittance and bandgap, resistivity, charge carrier concentration and mobility, and elemental depth profiles.
GIXRD has been conducted using Rigaku - Smartlab. The x-ray source Cu K-alpha at 1.54 Å, the 2θ scans of the detector are from 15 to 65°, the step and the speed are 0.02° and 2°/min while the x-ray incident beam was kept at 0.55°. UV–Visible Spectroscopy was performed using Perkin Elmer - Lamda 1050, using 4 nm steps. Electrical properties were conducted for all samples using benchtop four-point probe system and Hall effect - Lakeshore 8400. Depth profiles were obtained using ToF-SIMS-IONTOF. Scanning/Transmission Electron Microscopy (SEM/TEM) images were obtained using FEI - Quanta 650/Talos, respectively. TEM lamella has been prepared by Focused Ion Beam (FIB)/SEM. The process consists of coating the SnOx film by a protective Pt layer. Then the whole stack Pt/SnO2/Substrate undergoes the thinning process to reach a convenient vertical thickness for the TEM imaging. TOF-SIMS analysis was performed by positive Bi+ ion primary beam at 30 keV and ~ 1.3 pA current over a 100 × 100 μm2 analysed area using random rastering mode while the sputtering was achieved using Cs+ ion beam at 2 keV over 400 × 400 μm2. The depth profile has been conducted in positive polarity which targets the positive ions emerging from the surface at each etched level.
Following a first visual observations just after the SnOx deposition, all the samples looked homogenous and most of the films had the yellowish colour which is a typical characteristic of SnOx material.
For the as-deposited SnOx at 100 °C, the samples do not show any XRD sharp peak, thereby indicating the low crystalline quality and/or the amorphous microstructure of this series (Fig. 1a). The sample deposited without O2 flow has a broad peak at around 30° revealing the presence of a very low crystalline microstructure of SnO. The XRD pattern for SnOx samples deposited in presence of O2 show a low crystalline SnO2 phase due to the shift of the broad peak from 30 to 34°. It is worth to note that the XRD pattern did not change while increasing the O2/Ar ratio from 0.5% to 7.5%, which may indicate that increasing the O2 flow does not improve the crystalline microstructure of SnOx at this deposition temperature of 100 °C.
GIXRD patterns of SnOx samples (a) as deposited (as-dep) at 100 °C, (b) as-dep at 250 °C, (c) as-dep at 100 °C and annealed in vacuum at 400 °C, (d) as-dep at 250 °C and annealed in vacuum at 400 °C, (e) as-dep at 100 °C and annealed in air at 400 °C, (f) as-dep at 250 °C and annealed in air at 400 °C.
For as-deposited SnOx at 250 °C (Fig. 1b), the samples deposited at O2/Ar ratios equal or above 0.5% have a XRD pattern which shows at least one peak, and the most intense peak located at 34° and corresponding to the (101) plane is observed for deposition at O2/Ar = 1.5%. This sample deposited at O2/Ar = 1.5% also exhibits the highest crystallite size corresponding to the (110) plane is 56 Å. The crystallite size decreases to 45 nm while increasing the O2/Ar from 1.5 to 4.5%. The crystallite size was not calculated for the other samples due to peak definition as shown in Table S2 (supplementary information). It is also expected that the growth conditions with the absence of O2 flow would favour the presence of SnO due to the material of the sputtering target. Furthermore, the growth under O2 flow has formed a SnO2 phase and hence, increasing the growth temperature has clearly improved the crystalline quality of SnO2 as concluded from XRD analysis.
For SnOx samples deposited at 100 °C and annealed at 400 °C under moderate vacuum, similarly to as-deposited samples at 100 °C without O2 flow, XRD pattern shows a broad peak at around 30° revealing the presence of low crystalline SnO for the sample deposited without O2 flow (Fig. 1c). The XRD pattern also shows a broad peak at around 34° related to the presence a low crystalline SnO2 phase for the samples deposited in the presence of O2. For O2/Ar above 0.5%, there is a small peak located at 34° and associated to the (101) plane. No noticeable change was then observed while varying the O2/Ar.
For SnOx samples deposited at 250 °C and annealed at 400 °C under moderate vacuum, similarly to previous samples deposited without O2 flow, there is a clear indication of a low crystalline SnO phase (Fig. 1d). While varying the O2/Ar from 0.5 to 7.5%, there is a clear crystalline microstructure as indicated by XRD pattern. The crystallite size related to SnO2 (110) peak decreases from 61 to 56 Å when O2/Ar goes from 1.5 to 2.5% and then it remains constant for higher O2/Ar ratios as revealed by Table S1 (supplementary information). It was clear that the vacuum annealing has improved the crystallinity of the SnOx samples deposited in presence of O2.
For SnOx samples deposited at 100 °C and annealed at 400 °C under air, all samples show a remarkable improved crystallinity (Fig. 1e). For air annealed SnOx deposited without O2 flow, there are two high crystalline phases of SnO and SnO2 as revealed by the presence of sharp peaks located at 29.9° indicating SnO and at 33.9° indicating SnO2. The crystallite size related to SnO2 (110) peak drastically decreases from 116 Å to 51 Å when O2/Ar goes from 0 to 0.5%. Then, it increases slightly up to 62 Å when O2/Ar varies from 0.5 to 2.5% and it decreases to 57 Å when O2/Ar goes from 2.5 to 7.5%. The sharp peak located at 26.6° and corresponding to the (110) plane of SnO2 deposited without O2, represents the highest crystallite size of all the deposited SnOx samples.
For SnOx samples deposited at 250 °C and annealed at 400 °C under air, all samples show an improved crystallinity compared to as-deposited SnOx (Fig. 1f). For air annealed SnOx deposited without O2 flow, there are also two high crystalline phases of SnO and SnO2 as revealed by the presence of sharp peaks located at 29.9° indicating SnO and at 33.9° indicating SnO2. The crystallite size of SnO2 drastically decreases from 109 Å to 54 Å when O2/Ar goes from 0 to 0.5%. Then, it slightly stabilizes around the value of 53 Å when O2/Ar goes from 0.5 to 7.5%. Air annealing has clearly improved the crystallinity of SnOx samples which were deposited at 100 °C compared to vacuum annealing. It is worth to note that SnO peaks were only observed in both air annealed samples deposited with O2 flow.
The as-deposited samples have shown that the relatively high temperature of 250 °C (i.e. as compared to 100 °C) has improved the crystalline microstructure for all the samples deposited with the presence of O2. This is due to the improved crystallization of SnOx and the reduction of the microstructure disorder31. Vacuum annealing has then slightly improved the crystallinity for samples deposited at 100 °C due to the higher annealing temperature of 400 °C. However, there is no clear improvement of the crystalline microstructure of the samples deposited at 250 °C. The air annealing has improved remarkably the SnOx microstructure for all samples deposited at 100 °C due to the higher annealing temperature of 400 °C, compared to the deposition temperature and to the abundant presence of O2 which enabled the crystallization of SnOx. Air annealing for SnOx sample deposited at 250 °C without O2 has improved substantially the crystallinity of SnOx due to the presence of O2 at higher temperature of 400 °C. Moreover, further crystallization was less extensive under air annealing for other samples deposited in presence of O2. This is likely due to the small temperature gradient between the deposition and the annealing processes, as well as the presence of O2 in both processes. SnO crystallinity was not achieved only by depositing SnO without O2 at both temperatures of 100 and 250 °C, as well as after annealing these samples under vacuum. However, SnO phase appeared in both samples deposited at 100 and 250 °C without O2 and annealed under air. This indicates that SnO crystallinity may be improved using a controlled annealing process under atmospheric pressure and in absence of O231,32,35,36,37,38. The different observations extracted from XRD are summarized in Table 1.
The electrical properties were investigated initially using the four-point probe IV tool then through the Hall effect measurements. Four-point probe measurements were performed on all samples using three-point statistics method. The obtained results revealed a very high resistivity around 108 Ω cm for SnOx deposited without the presence of O2 for both as-deposited series at 100 °C and 250 °C. As revealed by XRD results, this may be attributed to the low crystallinity of the SnOx films. The resistivity decreases to its lowest value of 47 Ω cm at O2/Ar = 0.5% for SnOx samples deposited at 100 °C and to its lowest value of 4.5 Ω cm at O2/Ar = 1.5% for SnOx samples deposited at 250 °C. The lower value of resistivity for samples deposited at 250 °C is matching with the highest crystallite size which indicated as discussed earlier the improvement of the crystalline microstructure.
For SnOx as deposited at 100 °C, the resistivity increases significantly from O2/Ar = 0.5 to 1.5% and it relatively stabilizes at higher O2/Ar starting from 1.5% (Fig. 2). For SnOx as deposited at 250 °C, the same behaviour is observed, where the resistivity increases significantly from O2/Ar = 1.5 to 2.5% and it relatively decreases towards higher O2/Ar starting from 2.5%. In presence of O2, all deposited SnOx samples at 250 °C show a better electrical conductivity compared to films grown at 100 °C.
Resistivity as a function of O2 flow in growth conditions for as-deposited, vacuum-annealed, and air-annealed samples.
Vacuum annealed samples were found to follow the similar pattern of as-deposited SnOx at both deposited temperatures where the best conductive as-deposited SnOx samples are still the best conductive ones. Moreover, the recorded resistivity values are 0.14 Ω cm for the best SnOx deposited at 100 °C and 0.07 Ω cm for the best SnOx deposited at 250 °C of all the fabricated batches after vacuum annealing. Furthermore, as expected after annealing process, all the recorded resistivity is significantly lower compared to the as-deposited ones. This improvement is likely attributed to the microstructure improvement and to the conservation of charge carrier after the vacuum annealing of the samples.
For both air annealed series, the lowest resistivity of around 0.13 Ω cm series was observed for samples deposited without O2 flow. The resistivity increases then significantly until O2/Ar = 1.5% and slightly stabilizes for higher O2/Ar starting from 1.5%. The trend of resistivity with respect to O2/Ar (of both air-annealed series) are very closely matching regardless the deposition temperature of SnOx. However, the resistivity for SnOx samples deposited at 250 °C is slightly lower for all O2/Ar ratios except the two extreme values of 0 and 7.5% as shown in Fig. 2.
The samples deposited at 250 °C followed by a moderate vacuum annealing at 400 °C showed the lowest resistivity of 0.07 Ω cm at O2/Ar = 1.5%. Furthermore, the other SnOx samples in the same series deposited with O2/Ar ratio above 1.5% show substantially a lower resistivity compared to samples from other series with the same conditions of O2/Ar. This can be attributed to the relatively higher crystallite size due to the annealing process as well as the expected charge carrier concentrations due to the vacuum annealing31,37,38.
Further analysis using Hall effect measurement were conducted solely on the best conductive samples identified by four-point probe method, both for vacuum and air annealed samples series. The electron mobility for the best conductive samples for each series were 1.07 cm2/V s for vacuum annealed series deposited at 100 °C, 7.77 cm2/V s for vacuum annealed series deposited at 250 °C, 2.11 cm2/V s for air annealed series deposited at 100 °C, and 2.58 cm2/V s for air annealed series deposited at 250 °C. Their respective charge carrier concentrations were 1.47 × 1019 cm−3 for vacuum annealed series deposited at 100 °C, 5.84 × 1018 cm−3 for vacuum annealed series deposited 250 °C, 1.39 × 1019 cm−3 for air annealed series deposited at 100 °C, and 1.26 × 1019 cm−3 for air annealed series deposited at 250 °C. All these conductive samples were n-type semiconductors which is expected for vacuum annealed samples due to the presence of SnO2 phase. All the results are summarised in Table 2.
However, the n-type conductivity for air annealed samples reveals that the majority charge carriers are related to the SnO2 phase. The most electrically conductive SnOx, which is deposited at 250 °C/O2/Ar = 1.5% and annealed under vacuum at 400 °C is a result of a much higher mobility and an average charge carrier concentration compared to other samples.
Normally, scattering mechanisms are the main explanation for the electron mobility. Furthermore, as per the grain-boundary scattering mechanism, the mobility increases while increasing the carrier concentration or the crystallite size. However, for higher values than 1020 cm−3 of charge carrier concentration, the mobility decreases due to the domination of ionised scattering mechanism36,39,40. Therefore, the grain-boundary scattering mechanism is likely the main mechanism responsible for the higher electron mobility of the best conductive SnOx sample. As a matter of fact, Kim et al.41 established that the grain boundary scattering was the dominant scattering mechanism for SnO2−x thin films prepared by magnetron sputtering41. In another work performed on polycrystalline GZO, Hall mobility measurements indicated that the mobility of electron which transports across many grains and grain boundaries in conduction path was limited by both scattering effects in ingrains and at grain boundaries42. The dominancy of the scattering effects varies with electron concentration. In the case of higher electron concentration above about 1020 cm−3, the dominant scattering effect for electron mobility (µHall) has been considered to be ingrain scattering (ionized-impurity scattering)43,44. On the other hand, it is well established that optical mobility (µopt) shows the electron mobility in ingrains. Thus, µopt is limited by the effect of ingrain scattering. In advanced investigations, comparing electron and optical mobilities has been employed to highlight the contribution of grain boundary scattering on electron mobility, and this approach has frequently been used as a means to study the effect of ingrain and grain boundary scattering on electron transport properties in many TCOs45,46,47,48,49,50.
The optical properties have been studied using UV–Visible spectroscopy. The optical transmittance measurements were conducted on all the grown samples and the average optical transmittance between 400 and 700 nm is summarized in Table 3. The average optical transmittance of the reference Quartz substrate was initially measured at 93%. For SnOx samples deposited at 100 °C, it is observed that the average optical transmittance from 400 to 700 nm (labelled transmittance) increases from 72 up to 89% when O2/Ar increases from 0 to 7.5%. Moreover, the transmittance increases from 70 to 89% after vacuum annealing and increases from 65 to 91% when O2/Ar varies from 0 to 7.5% after air annealing. For SnOx samples deposited at 250 °C, the transmittance in as-deposited and vacuum annealed samples decreases from around 80% to 76% when O2/Ar varies from 0% to 0.5%. Furthermore, the transmittance increases up to 91% while O2/Ar increasing from 0.5% to 7.5%. The transmittance increases from 77 to 91% while O2/Ar varying from 0 to 7.5% after air annealing. These results are summarised in Table 3.
It is established that the oxygen flow has a strong effect on the optical properties of SnOx36. This can be directly observed by the blue shift of the absorption edge as well as the relative increase of transmittance when O2/Ar is increasing as shown in Figure S1 (supplementary information).
Figure S2 (supplementary information) show Tauc plots for all samples considering the SnOx thin films as a direct bandgap semiconductor. It can be concluded from Tauc plots that all the SnOx films have a wide optical band gap varying from 3.3 to 4.5 eV. Table 4 summarised the values of the optical band gap for all the samples, which are found to increase and then stabilise when O2/Ar is increasing. The low optical bandgap for the SnOx samples deposited without the presence of O2 compared to the other samples, is a typical characteristic for SnO and it was already reported in the literature varying from 2.6 to 3.4 eV36. These results corroborate well the XRD analysis and are confirming the major presence and the low crystallinity behaviour of the SnO phase for both the as-deposited and vacuum annealed samples. The measured bandgap above 4 eV for the rest of the samples is related to SnO2 phase which is very close to the values reported in the literature. The band gap variation is mainly related to the difference of the stoichiometry of the SnOx films. However, the effect of the sample disorder can also decrease the bandgap of the SnOx thin films35,36,51.
The band gap of SnO2 samples prepared in presence of O2 is relatively very high compared to the literature where all samples reached 4.4–4.5 eV except one sample of SnO2 deposited at 100 °C/0.5% O2:Ar and annealed under vacuum. These band gap values are related to the high oxidation of SnO2 due to the oxidized sputtering target and the presence of oxygen during the growth.
The optoelectronic performance for all SnOx samples was evaluated using Haacke figure of merit (FoM) Eq. (1)52. The results are shown in Table 5. SnOx samples have a relatively low figure of merit due to the moderate resistivity of the thin films. The highest figure of merit φ of 5.14 × 10–2 (10–3 Ω−1) is related to our best conductive SnOx sample. Furthermore, the SnOx samples deposited at 250 °C where the O2/Ar ratio between 1.5 and 4.5% as well as SnOx sample deposited at 100 °C and at 0.5% O2/Ar ratio have shown figure of merit above 10–2 (10–3 Ω−1). These results reveal that the vacuum annealing has improved the optoelectronic performance of the SnOx thin films.
where φ is figure of merit, T is the average optical transmittance from 400 to 700 nm, and Rs is the sheet resistance.
Table S3 (supplementary information) summarizes selected values from relevant literature of FoM for different doped SnO2 thin films along with the doping type, synthesis method, band gap value, electrical resistivity and sheet resistivity, and optical transmittance53,54,55,56,57,58,59,60,61,62,63,64,65,66,67,68,69,70,71,72,73,74,75. Only two references of undoped films has been found in addition to our present work. Figure 3 highlights these FoM and band gap values as a function of the various references along with our present work. Highest FoM has been recorded for SnO2 grown with spray pyrolysis and doped with fluorine, while the lowest value characterized SnO2 deposited by Pulsed Laser Deposition and doped with Tellurium. While our measured FoM belongs rather to the category of low values in Table S3 (supplementary information), which is rather expected since our SnO2 films are undoped and did not reach a low resistivity around 10–4 Ω cm. However, the optical band gap was among the highest reported in the literature data which is, as discussed previously, due to the high oxidation state of SnOx.
Summary of Figure of Merit values and bang gap as a function of the various references53,54,55,56,57,58,59,60,61,62,63,64,65,66,67,68,69,70,71,72,73,74,75.
SEM images were conducted on the four samples from the annealed series showing the highest conductivity as well as their related SnOx samples without annealing. All four samples show a crack-free SnOx films. The annealing process did not change drastically the morphology of the SnOx thin films. It can be clearly observed that the best conductive sample deposited at 250 °C with O2/Ar = 1.5% and annealed under vacuum (Fig. 4) has the largest grain size compared to the other samples as shown in Fig. 4. The large grain size has improved the conductivity of SnOx as previously reported36. Following the discussion regarding the Hall effect results, the high mobility associated to these specific growth conditions is also attributed to the large grain size33,36. The sample deposited at 100 °C and with O2/Ar = 0.5% and annealed under vacuum (Fig. 4c) has shown the smallest grain size compared to the other three SnOx samples. This result is corroborating well the Hall effect measurement as it has shown the lowest mobility among the four selected samples.
Representative SEM images for the most electrically conductive annealed samples and their related SnOx samples without annealing: (a) SnOx deposited at 100 °C, 0.5% O2/Ar, annealed under vacuum, (b) SnOx deposited at 100 °C, 0.5% O2/Ar, without annealing, (c) SnOx deposited at 250 °C, 1.5% O2/Ar, annealed under vacuum, (d) SnOx deposited at 250 °C, 1.5% O2/Ar, without annealing, (e) SnOx deposited at 100 °C, 0% O2/Ar, annealed in air, (f) SnOx deposited at 100 °C, 0% O2/Ar, without annealing, (g) SnOx deposited at 250 °C, 0% O2/Ar, annealed in air, (h) SnOx deposited at 250 °C, 0% O2/Ar, without annealing.
In order to confirm the multi-crystalline structure of the best conductive SnO2 sample, we performed TEM imaging and mapping (Fig. 5). The interplanar spacing could be measured directly from the image (Fig. 5a) namely (110) and (101) planes, which is matching with the results revealed by XRD. Figure 5b shows the TEM diffraction pattern indexation revealing the intense patterns are related to (110) and (101) planes. High-angle annular dark-field imaging (HAADF) shown in Fig. 5c has revealed a dense SnO2 film with elongated column-shape crystalline structure towards the growth direction. EDS mapping (Fig. 5d) has revealed the presence of a uniform layer of SnO2 which is forming a sharp and clear interfaces with the quartz substrate33,36.
Cross-section TEM images for the best conductive sample (a) high resolution TEM image, (b) TEM diffraction pattern, (c) HAADF image, (d) EDS mapping.
After having investigated the structural properties with XRD and TEM, we further confirm the homogeneity of the SnOx thin film by performing TOF-SIMS on the best conductive sample to show the presence of high quality SnOx by the two constant intensities of Sn and O in the ToF-SIMS steady state conditions between the surface and the interface as shown in Fig. 6. This analysis reveals the constant stoichiometry throughout the depth. The ion yield is much higher for Sn at the surface and the interface due to the matrix effect where the chemical environment changes as the secondary ion yields are strongly dependent on the chemical environment, which explains the high intensity of Sn at the surface and the interface. Si intensity is also much higher at the surface due to a combination of some surface contamination and the higher ion yield at the surface as described previously. SIMS in general is inherently not a quantitative measurement technique. The secondary ion yields are strongly dependent on the chemical environment (matrix effect) and therefore, there is no direct correlation of elemental/compound intensity vs concentration. This technique also revealed the presence of H which slightly increases from the surface to the interface76. TOF-SIMS also confirmed the absence of organic or inorganic contamination throughout the depth and it shows also perfect interfaces between the SnOx thin film and the quartz substrate.
TOF-SIMS profiles for the best conductive sample (SnOx deposited at 250 °C, at 1.5% O2/Ar ratio, and annealed under vacuum at 400 °C).
In summary, for as deposited SnOx and based on the characterization and discussion, it is suggested that SnOx is predominantly amorphous and/or showing low crystalline SnO in absence of O2, owing to the nature of the sputtering target (i.e. SnO). This has led to a lower electrical conductivity. At low O2/Ar ratios, the predominating phase become SnO2 and the crystallinity tended to improve at higher temperature of 250 °C as the O2/Ar ratio increased from 0.5 to 1.5%. The poor oxygen condition is suggested to form defects within SnOx thin film particularly oxygen vacancies and these defects are expected to decrease while increasing the O2/Ar ratio. This has led to a higher electrical conductivity. At higher O2/Ar ratios, the crystallinity decreased at higher temperature of 250 °C. The rich O2 condition is expected to reduce the oxygen vacancy defects and eventually decreases the charge carrier concentration. This has led to a lower electrical conductivity. Both annealing processes are expected to improve the crystallinity of the films due to the thermal treatment at 400 °C for 1 h. However, vacuum annealing is expected to conserve the charge carriers concentrations by preventing annihilation of the oxygen vacancies due to the lack of O2. On the other hand, air annealing is expected to reduce the charge carriers concentrations by filling the oxygen vacancies with oxygen supplied from air.
The air annealing has shown a better crystallinity compared to vacuum annealing as all the samples have reported high crystallinity and two SnOx samples deposited without O2 recorded the highest crystallite size. Both SnOx deposited without O2 and annealed in air have revealed that the presence of both phases SnO and SnO2, and it is clear that O2 from the air atmosphere has oxidized significantly SnO to SnO2. Moreover, as per crystallite size Table S2, we found that the reported crystallite size is relatively higher after vacuum annealing the SnOx samples (deposited at 250 °C). While for the annealing under vacuum (i.e. absence of O2), the charge carrier concentration which is mainly due to O vacancies is expected to be conserved. The combination of improved crystallite size and the conservation of charge carrier are the key factors for improving the electrical conductivity as compared to SnOx samples from other series with same O2/Ar ratio.
Our study highlighted the structure/performance correlations of SnOx thin films grown by RF MS. High quality SnOx samples were prepared using magnetron sputtering deposition method followed by thermal annealing processes. Crystalline microstructure, electrical and optical properties were characterised in-depth. Both SnO2 and mixed SnO/SnO2 thin films were synthetized using RF sputtering. The most electrically conductive sample was obtained by using O2/Ar = 1.5% during the growth at 250 °C followed by a moderate vacuum post annealing at 400 °C/5 × 10–4 Torr, and has shown a compact and dense morphology without presence of pinholes or cracks, and its grain size were relatively larger compared to other samples, which clearly improved the electron mobility. Its average optical transmittance between 400 and 700 nm was measured to be above 80%. The best optical transmittance of 91% is achieved only using the highest O2/Ar ratio of 7.5% for deposited SnOx at 250 °C without annealing and with vacuum annealing as well as both air annealed samples. Vacuum annealing provided a higher electrical conductivity compared to the as-deposited and air-annealed processes. This is attributed to the improvement of crystalline microstructure as well as the presence of oxygen lattice vacancies which has led to a high charge carrier concentration. These growth conditions summarise a good compromise between a high grain size, higher crystalline structure, and high charge carrier concentration.
The data are available from the corresponding author upon a reasonable request.
Lewis, B. G. & Paine, D. C. Applications and processing of transparent conducting oxides. MRS Bull. 25(8), 22–27 (2000).
Ellmer, K., Mientus, R. & Seeger, S. Metallic oxides (ITO, ZnO, SnO2, TiO2). In Transparent Conductive Materials (eds Levy, D. & Castellón, E.) 31–80. https://doi.org/10.1002/9783527804603 (Wiley-VCH, 2018).
Nathan, A., Lee, S., Jeon, S., Song, I. & Chung, U.-I. Transparent oxide semiconductors for advanced display applications. Inf. Displ. 29(1), 6–11 (2013).
Jang, J., Kang, Y., Cha, D., Bae, J. & Lee, S. Thin-film optical devices based on transparent conducting oxides: Physical mechanisms and applications. Curr. Comput. Aided Drug Des. 9(4), 192 (2019).
Park, J. S., Maeng, W.-J., Kim, H.-S. & Park, J.-S. Review of recent developments in amorphous oxide semiconductor thin-film transistor devices. Thin Solid Films 520(6), 1679–1693 (2012).
Article ADS CAS Google Scholar
Hosono, H. 68.3: Invited paper: Transparent amorphous oxide semiconductors for high performance TFT. In SID Symposium Digest of Technical Papers, Vol. 38, no. 1 1830–1833 (2007).
Kamiya, T. & Hosono, H. Material characteristics and applications of transparent amorphous oxide semiconductors. NPG Asia Mater. 2(1), 15–22 (2010).
Nomura, K. et al. Room-temperature fabrication of transparent flexible thin-film transistors using amorphous oxide semiconductors. Nature 432(7016), 488–492 (2004).
Article ADS CAS PubMed Google Scholar
Nathan, A., Lee, S., Jeon, S. & Robertson, J. Amorphous oxide semiconductor TFTs for displays and imaging. J. Disp. Technol. 10(11), 917–927 (2014).
Article ADS CAS Google Scholar
Fujiwara, H. & Fujimoto, S. Transparent conductive oxide materials. In Spectroscopic Ellipsometry for Photovoltaics: Volume 1: Fundamental Principles and Solar Cell Characterization (eds Fujiwara, H. & Collins, R. W.) 523–563 (Springer, 2018).
de Andrés, A., Jiménez-Villacorta, F. & Prieto, C. The compromise between conductivity and transparency. In Transparent Conductive Materials (eds Levy, D. & Castellón, E.) 1–30. https://doi.org/10.1002/9783527804603.ch1 (Wiley-VCH, 2018).
Dalapati, G. K. et al. Tin oxide for optoelectronic, photovoltaic and energy storage devices: A review. J. Mater. Chem. A 9(31), 16621–16684 (2021).
Scanlon, D. O. & Watson, G. W. On the possibility of p-type SnO2. J. Mater. Chem. 22(48), 25236–25245 (2012).
Jiang, Q., Zhang, X. & You, J. SnO2: A wonderful electron transport layer for perovskite solar cells. Small 14(31), 1801154 (2018).
Fukai, Y., Kondo, Y., Mori, S. & Suzuki, E. Highly efficient dye-sensitized SnO2 solar cells having sufficient electron diffusion length. Electrochem. Commun. 9(7), 1439–1443 (2007).
Calderer, J. et al. Synthesis and characterisation of metal suboxides for gas sensors. Microelectron. Reliab. 40(4), 807–810 (2000).
Coles, G. S. V., Williams, G. & Smith, B. The effect of oxygen partial pressure on the response of tin (IV) oxide based gas sensors. J. Phys. D Appl. Phys. 24(4), 633–641 (1991).
Article ADS CAS Google Scholar
Liang, L. Y. et al. Phase and optical characterizations of annealed SnO thin films and their p-type TFT application. J. Electrochem. Soc. 157(6), H598 (2010).
Ogo, Y. et al. p-channel thin-film transistor using p-type oxide semiconductor, SnO. Appl. Phys. Lett. 93(3), 032113 (2008).
Article ADS CAS Google Scholar
Isono, T. et al. Highly conductive SnO2 thin films for flat-panel displays. J. Soc. Inf. Disp. 15(2), 161–166 (2007).
Odani, A. et al. Development and testing of nanomaterials for rechargeable lithium batteries. J. Power Sources 119–121, 517–521 (2003).
Han, Z. et al. Solvothermal preparation and morphological evolution of stannous oxide powders. Mater. Lett. 48(2), 99–103 (2001).
Liu, A., Zhu, M. & Dai, B. A novel high-performance SnO2 catalyst for oxidative desulfurization under mild conditions. Appl. Catal. A 583, 117134 (2019).
Pan, X. Q. & Fu, L. Oxidation and phase transitions of epitaxial tin oxide thin films on (1̄012) sapphire. J. Appl. Phys. 89(11), 6048–6055 (2001).
Article ADS CAS Google Scholar
Fan, H. & Reid, S. A. Phase transformations in pulsed laser deposited nanocrystalline tin oxide thin films. Chem. Mater. 15(2), 564–567 (2003).
Kim, K.-K., Kim, H.-S., Hwang, D.-K., Lim, J.-H. & Park, S.-J. Realization of p-type ZnO thin films via phosphorus doping and thermal activation of the dopant. Appl. Phys. Lett. 83(1), 63–65 (2003).
Article ADS CAS Google Scholar
Zhang, T. et al. Atomic layer deposited ZnxNi1−xO: A thermally stable hole selective contact for silicon solar cells. Appl. Phys. Lett. 113(26), 262102 (2018).
Article ADS CAS Google Scholar
Bosman, A. J. & Crevecoeur, C. Mechanism of the electrical conduction in Li-doped NiO. Phys. Rev. 144(2), 763–770 (1966).
Article ADS CAS Google Scholar
Hossain, M. A. et al. Doped nickel oxide carrier-selective contact for silicon solar cells. IEEE J. Photovolt. 11(5), 1176–1187 (2021).
Pollack, G. P. & Trivich, D. Photoelectric properties of cuprous oxide. J. Appl. Phys. 46(1), 163–172 (1975).
Article ADS CAS Google Scholar
Kim, C., Kim, S. & Kim, S. E. Transparent SnOx thin films fabricated by radio frequency reactive sputtering with a SnO/Sn composite target. Thin Solid Films 634, 175–180 (2017).
Article ADS CAS Google Scholar
Sundqvist, J., Lu, J., Ottosson, M. & Hårsta, A. Growth of SnO2 thin films by atomic layer deposition and chemical vapour deposition: A comparative study. Thin Solid Films 514(1), 63–68 (2006).
Article ADS CAS Google Scholar
Jiang, J. C., Lian, K. & Meletis, E. I. Influence of oxygen plasma treatment on the microstructure of SnOx thin films. Thin Solid Films 411(2), 203–210 (2002).
Article ADS CAS Google Scholar
Guo, W. et al. Microstructure, optical, and electrical properties of p-type SnO thin films. Appl. Phys. Lett. 96(4), 042113 (2010).
Article ADS CAS Google Scholar
Jarzebski, Z. M. & Morton, J. P. Physical properties of SnO2 materials. III. Optical properties. J. Electrochem. Soc. 123(10), 333C–46C (1976).
Article ADS CAS Google Scholar
Tao, Y., Zhu, B., Yang, Y., Wu, J. & Shi, X. The structural, electrical, and optical properties of SnO2 films prepared by reactive magnetron sputtering: Influence of substrate temperature and O2 flow rate. Mater. Chem. Phys. 250, 123129 (2020).
Khan, A. F., Mehmood, M., Rana, A. M. & Bhatti, M. T. Effect of annealing on electrical resistivity of rf-magnetron sputtered nanostructured SnO2 thin films. Appl. Surf. Sci. 255(20), 8562–8565 (2009).
Article ADS CAS Google Scholar
Sethi, R., Ahmad, S., Aziz, A. & Siddiqui, A. M. Structural, optical and electrical properties of tin oxide thin films for application as a wide band gap semiconductor. AIP Conf. Proc. 1675(1), 030039 (2015).
Zhu, B. et al. Characteristics of Al-doped ZnO thin films prepared in Ar + H2 atmosphere and their vacuum annealing behavior. J. Vac. Sci. Technol. A 31(6), 061513 (2013).
Zhu, B. L. et al. Investigation of structural, electrical, and optical properties for Al-doped ZnO films deposited at different substrate temperatures and H2 ratios. J. Electrochem. Soc. 159(5), H536–H544 (2012).
Kim, I. H. et al. Scattering mechanism of transparent conducting tin oxide films prepared by magnetron sputtering. Thin Solid Films 515(4), 2475–2480 (2006).
Article ADS CAS Google Scholar
Yamada, T., Makino, H., Yamamoto, N. & Yamamoto, T. Ingrain and grain boundary scattering effects on electron mobility of transparent conducting polycrystalline Ga-doped ZnO films. J. Appl. Phys. 107(12), 123534 (2010).
Article ADS CAS Google Scholar
Steinhauser, J., Faÿ, S., Oliveira, N., Vallat-Sauvain, E. & Ballif, C. Transition between grain boundary and intragrain scattering transport mechanisms in boron-doped zinc oxide thin films. Appl. Phys. Lett. 90(14), 142107 (2007).
Article ADS CAS Google Scholar
Fujiwara, H. & Kondo, M. Effects of carrier concentration on the dielectric function of ZnO: Ga and In2O3:Sn studied by spectroscopic ellipsometry: Analysis of free-carrier and band-edge absorption. Phys. Rev. B 71(7), 075109 (2005).
Article ADS CAS Google Scholar
Yamamoto, T., Sakemi, T., Awai, K. & Shirakata, S. Dependence of carrier concentrations on oxygen pressure for Ga-doped ZnO prepared by ion plating method. Thin Solid Films 451–452, 439–442 (2004).
Brehme, S. et al. Free-carrier plasma resonance effects and electron transport in reactively sputtered degenerate ZnO: Al films. Thin Solid Films 342(1), 167–173 (1999).
Article ADS CAS Google Scholar
Hamberg, I. & Granqvist, C. G. Evaporated Sn-doped In2O3 films: Basic optical properties and applications to energy-efficient windows. J. Appl. Phys. 60(11), R123–R160 (1986).
Article ADS CAS Google Scholar
Volintiru, I., Creatore, M. & van de Sanden, M. C. M. In situ spectroscopic ellipsometry growth studies on the Al-doped ZnO films deposited by remote plasma-enhanced metalorganic chemical vapor deposition. J. Appl. Phys. 103(3), 033704 (2008).
Article ADS CAS Google Scholar
Ellmer, K. Resistivity of polycrystalline zinc oxide films: Current status and physical limit. J. Phys. D Appl. Phys. 34(21), 3097–3108 (2001).
Article ADS CAS Google Scholar
Minami, T., Sato, H., Ohashi, K., Tomofuji, T. & Takata, S. Conduction mechanism of highly conductive and transparent zinc oxide thin films prepared by magnetron sputtering. J. Cryst. Growth 117(1), 370–374 (1992).
Article ADS CAS Google Scholar
Manifacier, J. C., De Murcia, M., Fillard, J. P. & Vicario, E. Optical and electrical properties of SnO2 thin films in relation to their stoichiometric deviation and their crystalline structure. Thin Solid Films 41(2), 127–135 (1977).
Article ADS CAS Google Scholar
Haacke, G. New figure of merit for transparent conductors. J. Appl. Phys. 47(9), 4086–4089 (1976).
Article ADS CAS Google Scholar
Fauzia, V., Yusnidar, M. N., Lalasari, L. H., Subhan, A. & Umar, A. A. High figure of merit transparent conducting Sb-doped SnO2 thin films prepared via ultrasonic spray pyrolysis. J. Alloy. Compd. 720, 79–85 (2017).
Islam, M. A., Mou, J. R., Hossain, M. F. & Hossain, M. S. Highly transparent conducting and enhanced near-band edge emission of SnO2: Ba thin films and its structural, linear and nonlinear optical properties. Opt. Mater. 106, 109996 (2020).
Hossain, M. F., Shah, M. A. H., Islam, M. A. & Hossain, M. S. Transparent conducting SnO2 thin films synthesized by nebulized spray pyrolysis technique: Impact of Sb doping on the different physical properties. Mater. Sci. Semicond. Process. 121, 105346 (2021).
Ramarajan, R., Kovendhan, M., Thangaraju, K. & Paul, J. D. Substrate temperature dependent physical properties of spray deposited antimony-doped SnO2 thin films. Thin Solid Films 704, 137988 (2020).
Article ADS CAS Google Scholar
Ramarajan, R. et al. Large-area spray deposited Ta-doped SnO2 thin film electrode for DSSC application. Sol. Energy 211, 547–559 (2020).
Article ADS CAS Google Scholar
Sivakumar, P. et al. Influence of Ga doping on structural, optical and electrical properties of transparent conducting SnO2 thin films. Optik. 226, 165859 (2021).
Article ADS CAS Google Scholar
Sivakumar, P. et al. Effect of Ti doping on structural, optical and electrical properties of SnO2 transparent conducting thin films deposited by sol-gel spin coating. Opt. Mater. 113, 110845 (2021).
Tarighi, A. & Mashreghi, A. Dependence of photovoltaic properties of spray-pyrolyzed F-doped SnO2 thin film on spray solution preparation method. J. Electron. Mater. 48(12), 7827–7835 (2019).
Article ADS CAS Google Scholar
Tran, Q.-P., Fang, J.-S. & Chin, T.-S. Properties of fluorine-doped SnO2 thin films by a green sol–gel method. Mater. Sci. Semicond. Process. 40, 664–669 (2015).
Li, B. et al. Influences of ultrasonic vibration on morphology and photoelectric properties of F-doped SnO2 thin films during laser annealing. Appl. Surf. Sci. 458, 940–8 (2018).
Article ADS CAS Google Scholar
Chan y Díaz, E., Camacho, J. M., Duarte-Moller, A., Castro-Rodríguez, R. & Bartolo-Pérez, P. Influence of the oxygen pressure on the physical properties of the pulsed-laser deposited Te doped SnO2 thin films. J. Alloys Compd. 508(2), 342–7 (2010).
Mrabet, C., Boukhachem, A., Amlouk, M. & Manoubi, T. Improvement of the optoelectronic properties of tin oxide transparent conductive thin films through lanthanum doping. J. Alloys Compd. 666, 392–405 (2016).
Joseph, D. P., Renugambal, P., Saravanan, M., Raja, S. P. & Venkateswaran, C. Effect of Li doping on the structural, optical and electrical properties of spray deposited SnO2 thin films. Thin Solid Films 517(21), 6129–6136 (2009).
Article ADS CAS Google Scholar
Talaty, N. N., Beck, K., Citeau, H., Kirschbaum, K. & Giolando, D. M. Characterization of Tin(IV) oxide thin films prepared by atmospheric pressure chemical vapor deposition of cis-[SnCl4{OC(H)OC2H5}2]. Z. Anorg. Allg. Chem. 635(1), 53–63 (2009).
Moholkar, A. V., Pawar, S. M., Rajpure, K. Y. & Bhosale, C. H. Effect of concentration of SnCl4 on sprayed fluorine doped tin oxide thin films. J. Alloys Compd. 455(1), 440–446 (2008).
Ramarajan, R. et al. Boltzmann conductivity approach for charge transport in spray-deposited transparent Ta-doped SnO2 thin films. J. Alloys Compd. 897, 163159 (2022).
Moholkar, A. V., Pawar, S. M., Rajpure, K. Y., Patil, P. S. & Bhosale, C. H. Properties of highly oriented spray-deposited fluorine-doped tin oxide thin films on glass substrates of different thickness. J. Phys. Chem. Solids 68(10), 1981–1988 (2007).
Article ADS CAS Google Scholar
El Radaf, I. M. & Abdelhameed, R. M. Surprising performance of graphene oxide/tin dioxide composite thin films. J. Alloys Compd. 765, 1174–1183 (2018).
Adjimi, A., Aida, M. S., Attaf, N. & Ocak, Y. S. Gadolinium doping effect on SnO2 thin films optical and electrical properties. Mater. Res. Express 6(9), 096405 (2019).
Article ADS CAS Google Scholar
Belayachi, W. et al. SnO2 Films elaborated by radio frequency magnetron sputtering as potential transparent conducting oxides alternative for organic solar cells. ACS Appl. Energy Mater. 5(1), 170–177 (2022).
Babar, A. R. et al. Physical properties of sprayed antimony doped tin oxide thin films: The role of thickness. J. Semicond. 32(5), 053001 (2011).
Article ADS CAS Google Scholar
Vishwakarma, S. R., Upadhyay, J. P. & Prasad, H. C. Physical properties of arsenic-doped tin oxide thin films. Thin Solid Films 176(1), 99–110 (1989).
Article ADS CAS Google Scholar
Singh, S. K. & Basu, S. Characterisation of conducting SnO2 layers deposited by modified spray pyrolysis technique. Mater. Chem. Phys. 20(4), 381–396 (1988).
Deline, V. R., Katz, W., Evans, C. A. Jr. & Williams, P. Mechanism of the SIMS matrix effect. Appl. Phys. Lett. 33(9), 832–5 (1978).
Article ADS CAS Google Scholar
Authors are gratefully acknowledging the HBKU-Qatar Environment and Energy Research Institute (QEERI), Hamad Bin Khalifa University (HBKU), Qatar Foundation. We also acknowledge the support of Core Labs in QEERI namely, Pasha, Akshath, Atef and Jana for their technical contribution as well as Stephane Roques from ICube Laboratory. Authors are grateful for Qatar National Library (QNL), member of Qatar Foundation for the support in paying the Article Publication Charges (APC).
Qatar Environment and Energy Research Institute (QEERI), Hamad Bin Khalifa University (HBKU), Qatar Foundation, P.O. Box 34110, Doha, Qatar
Y. Zakaria, B. Aïssa, A. Samara & S. Mansour
ICube Laboratory - CNRS, University of Strasbourg, 67000, Strasbourg, France
Y. Zakaria, T. Fix, S. Ahzi & A. Slaoui
You can also search for this author in PubMed Google Scholar
You can also search for this author in PubMed Google Scholar
You can also search for this author in PubMed Google Scholar
You can also search for this author in PubMed Google Scholar
You can also search for this author in PubMed Google Scholar
You can also search for this author in PubMed Google Scholar
You can also search for this author in PubMed Google Scholar
V.B. designed the scheme and carried out the theoretical analysis under the guidance of F.O. V.B., F.O. reviewed the manuscript and contributed to the interpretation of the work and the writing of the manuscript.
Correspondence to B. Aïssa.
The authors declare no competing interests.
Springer Nature remains neutral with regard to jurisdictional claims in published maps and institutional affiliations.
Open Access This article is licensed under a Creative Commons Attribution 4.0 International License, which permits use, sharing, adaptation, distribution and reproduction in any medium or format, as long as you give appropriate credit to the original author(s) and the source, provide a link to the Creative Commons licence, and indicate if changes were made. The images or other third party material in this article are included in the article's Creative Commons licence, unless indicated otherwise in a credit line to the material. If material is not included in the article's Creative Commons licence and your intended use is not permitted by statutory regulation or exceeds the permitted use, you will need to obtain permission directly from the copyright holder. To view a copy of this licence, visit http://creativecommons.org/licenses/by/4.0/.
Zakaria, Y., Aïssa, B., Fix, T. et al. Study of wide bandgap SnOx thin films grown by a reactive magnetron sputtering via a two-step method. Sci Rep 12, 15294 (2022). https://doi.org/10.1038/s41598-022-19270-w
DOI: https://doi.org/10.1038/s41598-022-19270-w
Anyone you share the following link with will be able to read this content:
Sorry, a shareable link is not currently available for this article.
Provided by the Springer Nature SharedIt content-sharing initiative
By submitting a comment you agree to abide by our Terms and Community Guidelines. If you find something abusive or that does not comply with our terms or guidelines please flag it as inappropriate.
Scientific Reports (Sci Rep) ISSN 2045-2322 (online)
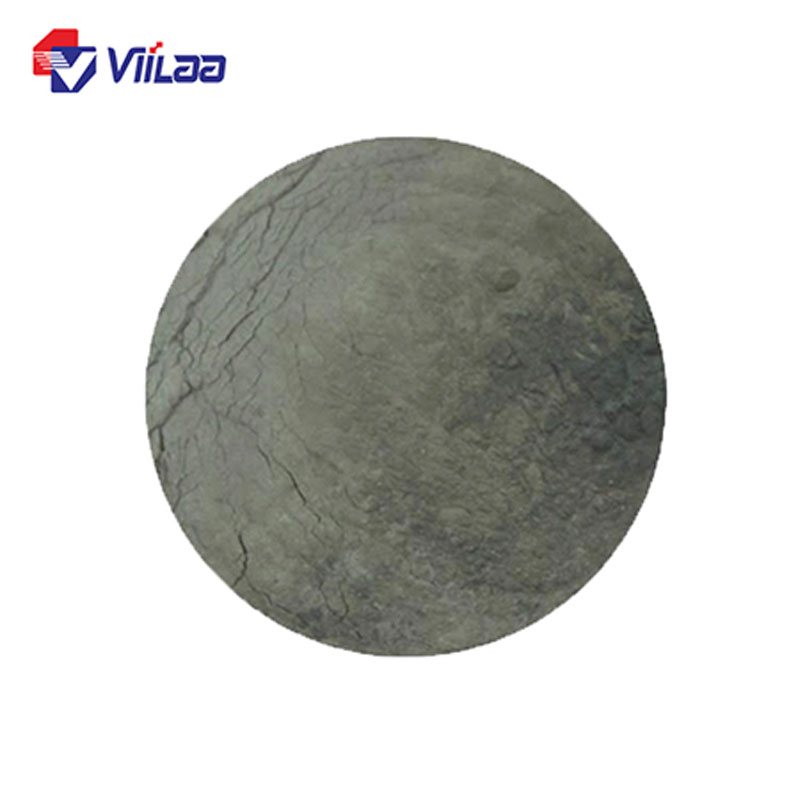
Yttrium Sputtering Target Sign up for the Nature Briefing newsletter — what matters in science, free to your inbox daily.